|
Introduction
The tuatara, or Sphenodon
Gray, 1831 (Günther 1867), is a reptile of about 300 mm snout to vent length (SVL) found on islands off the coast of New Zealand (Daugherty et al. 1990;
Cree et al. 1995;
Gaze 2001;
Parkinson 2002;
MacAvoy et al. 2007). For a long time many aspects of its anatomy were thought to represent the ancestral diapsid or even amniote condition (e.g.,
Cope 1896;
Byerly 1925;
von Wettstein 1931;
Romer 1956;
Sharell 1966), and it was therefore of great interest to comparative anatomists. This perception of Sphenodon led to many anatomical studies including those on the skeleton, muscles, major organs and vascular system (reviews include
von Wettstein 1931,
1932,
1937;
Robb 1977;
Dawbin 1982). More recent research has shown that many features previously considered to be "primitive" or plesiomorphic, such as the complete lower temporal bar, absence of an external ear, and reduced copulatory organ, are more parsimoniously interpreted as derived or secondary (Gans 1983;
Whiteside 1986;
Thompson and Daugherty 1992;
Evans 2003;
Böhme and Ziegler 2008;
Jones et al. 2009). Nevertheless, the phylogenetic position of Sphenodon as the only living member of Rhynchocephalia (Jones 2008), and therefore the only living sister taxon to Squamata (Rest et al. 2003;
Evans 2003;
Evans and Jones 2010), has meant that interest in Sphenodon anatomy remains high (e.g.,
Schwenk 1986,
2000;
Rieppel 1992;
Schmid et al. 1992;
Witmer 1995;
Reynoso 1996,
2003;
Herrel et al. 1998,
2007;
Reynoso and Clark 1998;
Reiner and Northcutt 2000;
Evans et al. 2001,
2002;
Alibardi and Maderson 2003;
Seligmann et al. 2003,
2008;
Meyer-Rochow et al. 2005;
Alibardi and Gill 2007;
Holliday and Witmer 2007,
2008;
Jones 2008,
2009;
Evans 2008;
Jones et al. 2009;
Kieser et al. 2009;
Johnston 2010). However, despite several descriptions of the skull (e.g.,
Günther 1867;
Siebenrock 1893,
1894;
Romer 1956;
Rieppel 1992;
Evans 2008;
Jones et al. 2009), the cranial joints have been largely overlooked.
Cranial Joint Structure
The skull is made up of bones that are united by fibrocellular joints at sutures (Pritchard 1956;
Kokich 1976;
Persson et al. 1978;
Cohen 2000;
Herring 2008). The term "suture" itself may be used to refer solely to the soft tissue component of a joint (e.g.,
Pritchard 1956;
Moss and Young 1960;
Bolt 1974;
Mao 2002) or more generally to the joint as a whole including the edges of bone that are involved (e.g.,
Jaslow 1990;
Cohen 2000). The exact histological structure of cranial joints may vary with age and location (Kokich 1976;
Wagemans et al. 1988;
Cohen 2000) but, in mammals at least, they are generally considered to comprise either three or five different tissue layers (Pritchard 1956;
Hinton 1988;
Cohen 2000;
Rafferty and Herring 1999;
Herring and Teng 2000). Each facial bone is surrounded by a layer of periosteum (an inner cambial layer and an outer capsular layer), and between the bones is a central vascular "middle space" spanned by collagen fibres (Figure 1; for abbreviations in figures see
Appendix 1) (Pritchard 1956). For cranial vault bones however the capsular layer and middle space are essentially absent. Instead the periosteum and dura converge into a central "ectomeninx" (Rafferty
and Herring 1999). The fibrocellular nature of cranial joints has prompted comparisons to the periodontal ligament that surrounds the tooth roots in mammals (e.g.,
Nanda and Hickory 1984;
Herring 2000).
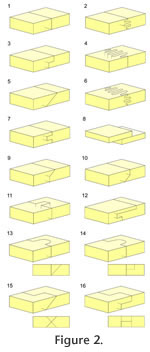
Here, 'facet' refers to the articulating surfaces of individual bones (following e.g.,
Evans 1980), 'seam' refers to the boundary between the bones in articulation (following
Kathe 1995,
1999), and 'interface' is used to refer to the articulation in cross-section.
Cranial joints can exhibit a variety of different constructions, and three main categories are recognised: butt joints, scarf joints and interdigitated joints. Butt joints (Figure 2.1), where bones meet at a flat wall perpendicular, or near perpendicular, to the outer surface of the bones (Moss 1957;
Bolt and Wassersug 1975;
Weishampel 1984;
Busbey 1995) are also sometimes termed vertical wall in
Kathe (1995), flat in
Bolt and Wassersug (1975), end-to-end in
Moss (1957) and
Wagemans et al. (1988). Scarf joints (Figure 2.5) involve a partial overlap between two bones (Weishampel 1984;
Busbey 1995) and may also be referred to as bevelled (Moss 1957;
Kathe 1995,
1999), overlapping (Moss 1957), shelved (Kathe 1995,
1999) or squamous (Moss 1957;
Bolt and Wassersug 1975). Interdigitated joints (Figure 2.2, 2.4 and 2.6) occur where processes from neighbouring bones interfinger and the externally visible seam may be complex and meandering (serrate in
Weishampel 1984).
These three categories are relatively easy to define, but difficult to apply without grouping together very differently shaped joints (Kathe 1995, p. 257;
Clack 2002). This problem is particularly true of overlapping and interdigitated joints. Variation in the degree and type of facet texture (e.g., gutters and striations or more conspicuous ridges, grooves and fluting) blur the boundaries between these three different joint categories. Moreover, bones do not always overlap in a simple slope-like fashion (Figure 2.5), the interface may be a stepped joint (Figure 2.10) or the overlapping bone may extend a tongue into an evenly recessed groove on the other bone (Figure 2.13-14).
Kathe (1995) recognised the problem of using only three categories and expanded their number, for example dividing scarf joints into 'shelf', 'basal shelf' and 'steep bevel' joints. He referred to the sloping facets of scarf joints as 'shelves', but the term 'shelf' is here considered more useful for describing rectangular planar projections of bone. Therefore, 'recessed scarf' is used here instead (Figure 2.10, 'butt-lap' of
Daza et al. 2008). A further overlapping joint type, referred to as a 'lap joint' by
Weishampel (1984), occurs where the facets are not inset (Figure 2.8).
Interdigitated joints also show a variety of forms depending on the number and shape of interdigitations, their depth from the cranial surface and their orientation. Problematically, the latter characteristic is not always clearly described. Here interdigitated joints are divided into three main categories:
• Type-A (Figure 2.2): several horizontal slots and shelves with facet surfaces generally parallel to the outer surface of the bone.
• Type-B (Figure 2.4): several vertical slots and projections with facets generally perpendicular to the outer surface of the bone.
• Type-C (Figure 2.6): spine-like projections overlapping in three planes, essentially a combination of Type A and Type B.
Type-A may be thought of as an exaggerated form of a slot contact (Figure 2.7). Similarly, Type-C interdigitations were described as "hypertrophied plug contacts" by Clack (2002). Some joints may contain radially distributed shelves of bone, for example the interfrontal or interparietal joint of some turtles (e.g.,
Kesteven 1910; MEHJ, pers. obs.), and involve a transition from Type-A to Type-B interdigitation within the same joint. Only Type-B and Type-C necessarily exhibit interdigitated external seams, and descriptions can be made more specific by reference to wavelength and amplitude (e.g.,
Figure 3;
Henderson 1998;
Clack 2002;
Rayfield 2005a).
Herring (1972, p. 224) used four ordinal categories to differentiate between seams of different complexity.
Previous Work on Cranial Joints
Joint morphology has been examined to assess the growth and functional morphology of the skull in a range of tetrapod taxa. To varying degrees this includes fish (e.g.,
Markey et al. 2005,
2006;
Markey and Marshall 2007a), 'lizards' (e.g.,
Frazzetta 1968;
Impey 1973;
Bell et al. 2003;
Evans 2008;
Daza et al. 2008;
Moazen et al. 2009;
Payne et al. 2011), amphisbaenians (e.g.,
Gans 1960,
1974), snakes (e.g.,
Rieppel 1978), turtles (e.g.,
Kesteven 1910;
Wegner 1959;
Gaffney 1979), crocodiles (e.g.,
Iordansky 1973;
Busbey 1995;
Cong et al. 1998;
Montero and Lessa 2000), birds (e.g.,
Bock 1964;
Cracraft 1968), cats (e.g.,
Buckland-Wright 1972;
Segura and Flores 2009), pigs and peccaries (e.g.,
Herring 1972,
1974;
Herring and Mucci 1991;
Rafferty and Herring 1999;
Herring and Teng 2000;
Herring et al. 2001;
Raffery et al. 2003;
Sun et al. 2004), goats (e.g.,
Jaslow 1989,
1990;
Jaslow and Biewner 1995;
Farke 2008), deer (Nicolay and Vaders 2006;
Slater et al. 2009;
Sánchez-Villagra 2010), rabbits (e.g.,
Persson et al. 1978,
1979;
Oudhof and Markens 1982;
Nash and Kokich 1985;
Bramble 1989;
Burrows et al. 1997;
Mao 2002;
Mao et al. 2003;
Radhakrishnan and Mao 2004;
Sarnat 2008), rodents (e.g.,
Moss 1954,
1957,
1961;
Miyawaki and Forbes 1987;
Gardner and Anderson 2001;
McLaughlin et al. 2000;
Byron et al. 2004,
2008;
Shibazaki et al. 2007;
Wilson and Sánchez-Villagra 2009), non-human primates (e.g.,
Beherents et al. 1978;
Nanda and Hickory 1984;
Wang et al. 2006) and humans (e.g.,
Kokich 1976;
Persson and Thilander 1977;
Persson et al. 1978;
Anton et al. 1992;
White 1996;
Margulies and Thibault 2000;
Sherick et al. 2000;
Morriss-Kay and Wilkie 2005;
Mann et al. 2009). Joints have also been considered in a growing number of fossil taxa, particularly Palaeozoic tetrapods (e.g.,
Bolt 1974;
Bolt and Wassersug 1975;
Klembara 1994;
Kathe 1995,
1999;
Thompson 1995;
Clack 2002;
Klembara et al. 2002;
Markey and Marshall 2007b) but also ornithopod dinosaurs (e.g.,
Weishampel 1984;
Norman and Weishampel 1985;
Rybczynski et al. 2008), theropod dinosaurs (e.g.,
Henderson 1998;
Currie et al. 2003;
Hurum and Sabath 2003;
Rayfield 2004,
2005b), mosasaurs (e.g.,
Baur 1892;
Callison 1967), plesiosaurs (e.g.,
Taylor 1992;
Taylor and Cruickshank 1993), choristoderes (Evans and Klembara 2005) and non-mammalian synapsids (e.g.,
Kemp 1972;
Jenkins et al. 2002;
Jasinoski et al. 2010a,
2010b).
Complete surveys of all the cranial joints in the skull are rare but examples include those carried out on modern pigs (Herring 1972), crocodiles (Iordansky 1973), ornithopod dinosaurs (Weishampel 1984) and a range of early tetrapods (e.g.,
Bolt and Wassersug 1975;
Kathe 1999;
Klembara 1994;
Clack 2002). Otherwise examinations tend to be more localised focused on one region of the skull in one taxon, such as the roofing bones (e.g.,
Sun et al. 2004;
Markey and Marshall 2007a), nasal-frontal (e.g.,
Rieppel 1978), frontal-zygomatic (jugal) (e.g.,
Kokich 1976) or zygomatic(jugal)-maxilla (e.g.,
Nanda and Hickory 1984). For recent reviews of cranial joints see
Herring (2000,
2008),
Cohen (2000),
Alaqeel et al. (2006) and
Depew et al. (2008).
Cranial Joint Function
Cranial joints are sites of bone growth (e.g.,
Oudhof 1982;
Koskinen et al. 1975;
Persson 1995;
Cohen 2000;
Opperman 2000;
Morriss-Kay 2001;
Mao 2002;
Sun et al. 2004;
Sarnat 2008). They are particularly important in humans that demonstrate a dramatic postnatal increase in brain volume, and premature closure of cranial joints can lead to abnormal growth and conditions such as craniosynostosis (e.g.,
Cohen and Kreiborg 1998;
Morriss-Kay and Wilkie 2005;
Adamo and Pollack 2009;
David et al. 2009). Nevertheless, growth of skull bones also occurs through internal and external surface remodelling (Brash 1934;
Enlow 1990) and surgical removal of cranial sutures in rabbits does not universally lead to an absence or even reduction of skull growth (Persson et al. 1979;
Sarnat 2008).
As well as being fundamental to growth, increasing evidence suggests that cranial joints also play an important role in skull mechanics (e.g.,
Gans 1960;
Buckland-Wright 1972;
Herring 1972;
Jaslow 1990;
Herring and Mucci 1991;
Rafferty et al. 2003;
Byron et al. 2004,
2008;
Markey et al. 2006;
Moazen et al. 2009), a hypothesis supported by several observations:
1. In many taxa a number of cranial joints remain open after adult size is attained (e.g.,
Moss 1961;
Herring 1972).
2. The fibrocellular nature of cranial joints means that they have very different material properties to those of the surrounding bone (e.g.,
Buckland-Wright 1972;
Jaslow 1990;
Herring 2000;
Margulies and Thibault 2000;
Radhakrishnan and Mao 2004;
Kupczik et al. 2007;
Moazen et al. 2009;
Jasinoski et al. 2010b). Correspondingly, they can respond very differently to stress, experiencing much higher levels of strain (e.g.,
Beherents et al. 1978;
Jaslow and Biewener 1995;
Rafferty and Herring 1999;
Herring and Teng 2000;
Thomason et al. 2001;
Markey et al. 2006). This observation has led to sutures being described as "shock absorbers" (Buckland-Wright 1972) and "strain sinks" (Rafferty et al., 2003) because the small movements they allow between bones may help to dissipate stress from the bones themselves. This role would be analogous to the expansion joints inserted between the concrete sections of buildings (McCormack 2006).
3. As discussed above, cranial joints can vary dramatically in shape both within a single taxon and between the skulls of different taxa with no obvious link to different growth regimes (e.g.,
Kathe 1995). The size and shape of cranial joints determines the amount of surface area available for soft tissue attachment (e.g.,
Gans 1960;
Herring 1972;
Jenkins et al. 2002). Therefore, more complex joints should be able to deal with greater stress (Buckland-Wright 1972). Scarf joints provide more surface area than butt joints but not as much as interdigitated joints (Herring 1972; Jaslow
1990). More complex joints also offer the potential for greater variation in orientation of the collagen fibres (Gans 1960;
Herring 1972;
Kemp 1972;
Jaslow 1990). Hence, interdigitated joints allow fibres to be arranged obliquely so as to resist compression (Herring
1972).
4. Highly complex sutures are often found in locations where high stress might be expected, for example, in the rostra of squamates and mammals
that use their head as a digging tool (e.g.,
Gans 1960,
1974;
Herring 1972;
Montero and Gans, 1999;
Kearney et al. 2005;
Daza et al. 2008), and around the horn cones of mammals that clash their heads during the rut (e.g., Jaslow 1989, 1990;
Jaslow and Biewener 1995;
Nicolay and Vaders 2006). Similarly, locations of high stress found in Finite Element Models of dinosaur skulls correspond to the position of complex or sliding joints (Rayfield 2004,
2005a).
5. Experiments on rats have shown that one month after surgery, neonates with both temporal muscles removed possess sagittal joints of relatively low complexity compared to control rats of similar age (Moss 1961). Also, myostatin-deficient mice that have hypertrophied temporal muscles and enhanced bite force compared to wild type mice also possess a sagittal joint seam of greater complexity and/or demonstrate greater squamosal-parietal overlap (Byron et al. 2004,
2008).
6. In vivo work on miniature pigs (Herring and Mucci 1991;
Rafferty and Herring 1999;
Herring and Teng 2000;
Rafferty et al. 2003) and fish (Markey and Marshall 2007a,
2007b) suggests that interdigitation is found at joints subject to compression, whereas simpler abutting scarf joints are associated with locations of tensile or torsonal forces. Note that this differs somewhat from suggestions by other researchers (e.g.,
Gans 1960;
Bolt and Wasserug 1975;
Thomson 1995;
Busbey 1995: 190) who considered butt contacts to be for compressive forces, overlapping joints for torsion and shear and interdigitated joints for tensile forces. Perhaps correspondingly,
Massler and Schour (1951) found that interdigitation may be the result of growth under tension. Alternatively,
Taylor (1992) suggested that butt joints, scarf joints and interdigitated joints represented a continuum of increased ability to withstand torsional forces. It is logical that an interdigitated joint can resist forces in a greater number of directions because of an increase in potential fibre orientations.
7. Histological examination shows that soft-tissue arrangements do appear to correspond with both overall cranial joint shape and local mechanical conditions (Buckland-Wright 1972;
Rafferty and Herring 1999;
Herring and Teng, 2000;
Sun et al. 2004). Therefore, butt-like joints incorporate connective tissues aligned to resist tension and interdigitated joints include soft tissues oriented obliquely to resist compression. Thus, the soft tissue component of cranial joints does not compensate for gross differences in skeletal anatomy.
8. The composition and stiffness of the soft tissue component is also subject to variation (e.g.,
Radhakrisham and Mao 2004) and, at least in some cases, this appears related to mechanical environment. Studies on rodents demonstrated that a decrease in mechanical loading led to a reduced secondary cartilage content of sutures (Hinton 1988). Connective tissue was also found to be more compliant in interparietal sutures experiencing higher tensile stress (Byron et al. 2004) and in vitro studies of rabbit cranial joints held in tension demonstrated increased synthesis of Type III collagen compared to control tissues (Meikle et al. 1984).
9. Finite element analysis of 3-D computer skull models with a representation of cranial joints suggests that these joints do alter local stress regimes and dissipate stress in the skull (Moazen et al. 2009).
Regardless of whether cranial joints play a mechanical role in skull function there is considerable evidence that the morphological appearance of cranial joints reflects their mechanical environment. For example, when sections of two different cranial joints (from mammals, e.g., rodents, rabbits) are swapped surgically (reciprocal translocation) in live animals each alters in time to resemble the original local joint morphology (e.g.,
Massler and Schour 1951;
Moss 1954,
1957;
Watanabe et al. 1957;
Markens and Oudhof 1980;
Oudhof 1982;
Oudhof and Markens 1982;
Nash and Kokich 1985). Similarly, sutures have been shown to alter or fuse in experimental animals and in explanted tissue specimens when stress is artificially increased or decreased (e.g.,
Moss 1961;
Meikle et al. 1979;
Nanda and Hickory, 1984;
Miyawaki and Forbes 1987;
Mao 2002,
Mao et al. 2003;
Heller et al. 2007). This
adaptability of cranial joints parallels the plastic response to mechanical stimuli widely observed in bone itself (e.g.,
Moore 1965;
Hinrichsen and Storey 1968;
Schumacher 1973;
Jones et al. 1977;
Lanyon 1980;
Corruccini and Beecher 1982;
Biewener and Bertram 1994;
Judex et al. 1997;
Lieberman 1997;
Hunt 1998;
Burr et al. 2002;
Boyde 2003;
Frost 2003).
Cranial joints probably also contain mechanoreceptors that provide feedback important for modulating muscle activity (Paphangkorakit and Osborn 1989), reducing the risk of damage to the feeding apparatus that may occur when excessive biting force is applied to hard food items. This potential function may be particularly important in Sphenodon which, unlike most mammals, does not have teeth held within sockets by periodontal ligament (Kieser et al. 2009;
Curtis et al. 2010a).
Cranial Joints and Kinesis in Sphenodon
In a seminal work of feeding in lepidosaurs
Schwenk (2000, p. 199) referred to cranial kinesis as "any intracranial mobility of the skull." However, as relative movements will occur between all cranial bones, albeit sometimes being very small (e.g.,
Behrents et al. 1978;
Buckland-Wright 1978;
Jaslow 1990;
Raffery and Herring 1999;
Herring and Teng 2000), a qualitative distinction between the presence or absence of cranial kinesis under this definition is arbitrary.
Rayfield (2005a) used the terms "active" and "passive" kinesis to distinguish between movements that occur during jaw loading and those generally larger movements associated with jaw opening and muscle contraction (such as those found in geckos,
Herrel et al. [1999]). Over the past hundred years different forms of "active" kinesis present in lepidosaurs (or at least squamates) have become defined (Table 1;
Evans 2008). The role of these various movements remains unclear but a number of possible advantages have been discussed including more effective prey acquistition, prey handling, prey transport, muscle leverage, tongue protrusion, tooth alignment, shearing, skull flattening, tooth disengagement and shock absorption (e.g.,
Frazzeta 1962;
Impey 1967;
Arnold 1998;
Herrel et al. 1999;
Schwenk 2000;
Metzger 2002;
Evans 2003,
2008;
Moazen et al. 2009).
The skull of Sphenodon is generally considered incapable of "active kinesis" (e.g.,
Günther 1867;
Versluys 1912;
Haas 1973;
Bellairs and Kamal 1981;
Gorniak et al. 1982;
Schwenk 2000;
Metzger 2002;
Evans 2008). This inference is largely based on the appearance of external suture seams or manipulation of articulated material (e.g.,
Ostrom 1962;
Arnold 1998).
Gorniak et al. (1982) did not observe any obvious skull kinesis during cineradiography of live animals feeding but admitted that the resolution was limited and none of the footage has been published. It has been suggested that limited metakinesis may be present in hatchling or juvenile Sphenodon (e.g.,
Howes and Swinnerton 1901;
Edgeworth 1935;
Ostrom 1962;
Arnold 1998). This hypothesis is based largely on the presence of constrictor dorsalis muscles, which run between the braincase and palate and are associated with metakinesis in squamates (e.g.,
Ostrom 1962;
Johnston 2010). Similarly, the presence of the protractor pterygoidei (part of the constrictor dorsalis) prompted
Lakjer (1926) and
Anderson (1936) to suggest that flexion between the front and back of the skull (akin to mesokinesis) might be possible. An alternative explanation for the presence of the constrictor dorsalis muscles is that they fullfil a proprioceptive role (to provide sensory feedback) and/or to support the skull during torsional strain (Evans 2008;
Johnston 2010).
Gardiner (1983, p. 50) referred to Sphenodon as possessing a "kinetic palate" but provided no clarification as to whether he considered this kinesis equivalent to hypokinesis or metakinesis.
Despite the widespread interest in lepidosaur cranial kinesis descriptions of internal joint structure in Sphenodon are few in number, are generally brief and often concentrate on the basipterygoid articulation (e.g.,
Howes and Swinnerton 1901;
Bolt 1974;
Arnold 1998;
Holliday and Witmer 2008;
Johnston 2010). Images are also limited, mainly comprising isolated figures of facets or joint overlaps that are not discussed in the text, for example, a disarticulated parietal and vomer in Siebenrock (1894), a squamosal in
Schauinsland (1903), a dotted line representing the overlap between the pterygoid and epipterygoid in
Lakjer (1926), dentaries in
Robb (1977, p. 14) and a photo of the exposed ventral surfaces of the palatines in
Sharrel (1966, p. 29). Information regarding the overlaps in hatchling Sphenodon are provided by the "exploded" skull (Figure 4) and cross-sections figured by
Howes and Swinnerton (1901, plate 6.1, 6.3) as well as the cleared-and-stained specimen described by
Rieppel (1992). Only recently have
Holliday and Witmer (2008) and
Johnston (2010) re-examined the basipterygoid articulation in any detail using computed tomography (CT).
Objectives
Here we present the first systematic survey of the cranial joints in Sphenodon with regard to the osteological component (Jones 2006,
2007). It will be used to assess the potential for skull kinesis and, in conjunction with observations of general skull structure, to generate hypotheses relating to skull mechanics (e.g.,
Herring 1972;
Buckland-Wright 1978;
Taylor 1992). This study will facilitate comparisons with fossil relatives of Sphenodon that are often known only from isolated skeletal material (e.g.,
Evans 1980;
Fraser 1982;
Whiteside 1986;
Säilä 2005; Jones 2006) as well as providing the basis for future computer modelling work (e.g.,
Moazen et al. 2009;
Curtis et al. 2010a,
2010b,
2010c).
|